Your cart is currently empty!
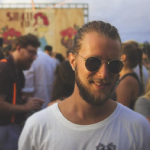
Williams Brown
Lorem ipsum dolor sit amet, consectetur adipisicing elit. Dolor, alias aspernatur quam voluptates sint, dolore doloribus voluptas labore temporibus earum eveniet, reiciendis.
Latest Posts
- Democrat at center of Kilmar Abrego Garcia saga responds to angel mom Patty Morin
- Secret Service Director Sean Curran offers behind-the-scenes look at what it takes to become an agent
- Girl, 14, killed by lion in Kenya
- Calls for US action amid growing violence against Christians in Nigeria
- Pope wishes worshippers Happy Easter after serious illness
Categories
Archive
Tags
Social Links
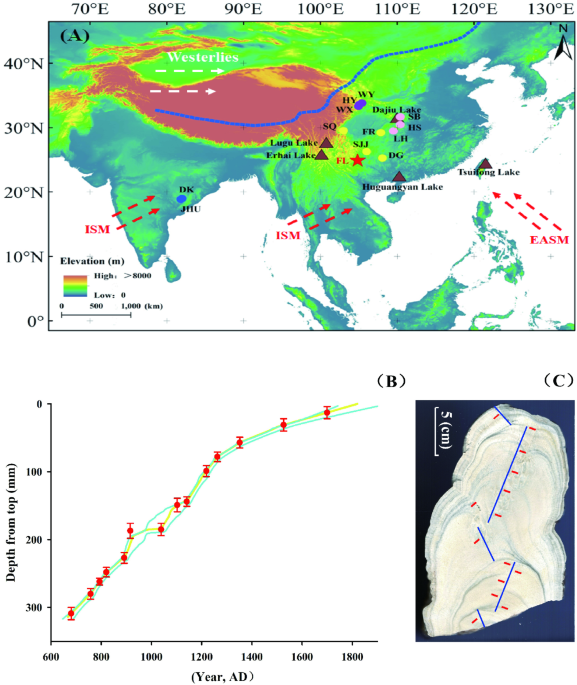
Significance of the stalagmite δ18O and δ13C
The δ18O of stalagmites in the Asian monsoon region are considered as indicators reflecting the strength of the ASM11,26,27,28,29,30,31. The stalagmite δ18O from Dongge Cave in Guizhou reflected the intensity of ASM variations on decadal to centennial scales under control of solar activity. It means that, the negative stalagmite δ18O values respond to stronger ASM, and vice versa11,31,32. Simulation results also indicated that the variation of stalagmite δ18O in the Asian monsoon region can reflect the intensity of ASM33. In addition, stalagmite δ18O is also influenced by various factors such as cave temperature, precipitation amount, winter/summer precipitation ratio, moisture source, moisture transport path, condensation and evaporation of moisture34,35,36. Stalagmite δ18O records accumulate the overall moisture changes from the moisture source to the cave, rather than indicating precipitation at a certain stage along the moisture transport path37,38. When the summer monsoon strengthens, whether it causes increasing in precipitation in upstream of moisture transport, i.e., strengthening of the “rain-out effect”, resulting in negative δ18O in downstream precipitation35; or directly enhances convective activity in downstream areas, leading to increased local precipitation. These scenarios can result in negative δ18O in precipitation, with consistent effects in both cases.
The δ18O precipitation values in Guiyang City, which is 327 km away from Feilong Cave, are influenced by the Asian monsoon. During summer (June–July–August: JJA), the δ18O values range from approximately − 10.5‰ to −16.8‰, while during winter (December-January-February: DJF), the range is about − 8.5‰ to −0.6‰ 18 (Supplementary Fig. 1) (https://websso.iaea.org/login/login.fcc). Summer precipitation accounts for over 80% of the total annual precipitation in this region. Therefore, stalagmite δ18O is mainly controlled by the δ18O of summer precipitation. The δ18O of stalagmites in this area reflects a comprehensive rainfall process between the summer monsoon water vapor source and the cave, rather than strictly corresponding to local precipitation amounts27,28. Whether the δ18O of stalagmites reflects changes in regional precipitation amounts needs to be comprehensively analyzed and cross-validated with other geochemical indicators, such as δ13C and trace elements39,40.
The δ13C of stalagmites is primarily influenced by both biotic and abiotic factors under climatic control41,42,43. Biotic factors include changes in vegetation type under temperature and precipitation control43,44, variations in overlying soil vegetation density or biomass, soil CO2 production rates, and microbial activity changes43,45, as well as changes in atmospheric CO2 concentration and sources46. Abiotic factors include hydrological conditions in karst systems, ventilation conditions, and kinetic fractionation during carbonate deposition processes47. The carbon in stalagmites mainly comes from atmospheric CO2, soil CO2, and the dissolution of surrounding carbonate rocks, with soil CO2 contributing the most (60–90%)43,48. The δ13C of stalagmites is mainly regulated by soil CO2. Soil CO2 dissolves in water to produce carbonic acid solution, which dissolves surrounding rocks along karst fissures, forming a mother solution rich in calcium carbonate. When the mother liquor enters the cave, CO2 degasses from dripping water (typically, pCO2 in dripping water is higher than in air), the solution becomes supersaturated, leading to calcium carbonate precipitation and stalagmite formation49,50.
Cave monitoring indicated that the δ13C of speleothems can reflect the vegetation and regional surface environmental conditions overlying cave. Therefore, stalagmite δ13C can indirectly reflect regional climatic conditions19,43,45,51. Additionally, enhanced human activities such as population migration, deforestation, and land reclamation can reduce the vegetation coverage above cave, weaken the respiration of plant roots and the decomposition of soil organic matter, lower soil microbial activity, decrease biological CO2 production, and cause stalagmite δ13C to shift positively19,39,40,43,52,53. In summary, there are uncertainties in using a single stalagmite proxy record to analyze past climate change. To accurately understand the climatic significance of stalagmite δ18O and δ13C records, comprehensive analysis combining other geochemical proxies and regional climate data is required for mutual verification39,40.
Variation of ASM during MWP and LIA
During the entire period from 770 to 1300 AD, the δ18O values of stalagmite FL2102 are lighter than the average of the entire stalagmite δ18O record (Fig. 2A), indicating an overall enhancement of the ASM during the MWP. However, after 1300 AD during the LIA, the δ18O values of stalagmite FL2102 are heavier than the average of the entire stalagmite δ18O record, indicating an overall weakening of the ASM (Fig. 2A).
During the MWP, comparing with stalagmite records from northern China, the record from Feilong Cave (this study) exhibits a certain similarity in trend with stalagmite δ18O records from Huangye Cave (105° 07′ E, 33° 35′ N)54, Wanxiang Cave (105° 00′ E, 33° 19′ N)55, and Wuya Cave (105° 25′ E, 33° 49′ N)56 (Fig. 3A–D). All four stalagmite δ18O records showed negative values compared to the baseline value of zero, indicating a strengthened ASM, along with a series of climate oscillations manifesting as decadal-scale positive δ18O excursions. Conversely, during the LIA, the δ18O values were predominantly positive relative to the baseline value of zero, indicating a weakened ASM11,31,39,40. The stalagmite record from Wuya Cave showed a sedimentary hiatus of nearly 500 years during the LIA (Fig. 3D)56.
Comparison with stalagmite records from China. (A) Feilong Cave (FL; this study); (B) Huangye Cave (HY)54; (C) Wanxiang Cave (WX)55; (D) Wuya Cave (WY)56; (E) Shijiangjun Cave (SJJ)57; (F) Furong Cave (FR)49; (G) Dongge Cave (DG)32; (H) Shenqi Cave (SQ)30. Dating points and errors for each record are represented by colored dots and error bars, respectively. Pink and gray bands represent the Medieval Warm Period and the Little Ice Age, respectively. The black dashed line denotes the benchmark value of zero in the Z-scores analysis of each record.
During the MWP, comparing with stalagmite records from southern China, δ18O records from Shijiangjun Cave (106° 03′ E, 26° 17′N)57, Furong Cave (107° 54′ E, 29°13′N)49, Dongge Cave (108° 05′ E, 25° 17′N)32and Shenqi Cave (103° 10′ E, 28° 93′ N)30 in southwest China show significant differences compared to the record from Feilong Cave (this study) (Fig. 3A,E,F,G,H). The δ18O values of stalagmites from these four caves did not exhibit stable negative deviations during the MWP. The most negative peak in stalagmite δ18O values from Shijiangjun Cave occurred around 1000 AD (Fig. 3E)57. In Furong Cave and Dongge Cave, the main period of negative δ18O values during the MWP is between 800 and 1000 AD (Fig. 3F,G)32,49. For Shenqi Cave, the most negative δ18O value was observed around 1250 AD (Fig. 3H)30. During the LIA, the stalagmite δ18O values from Shijiangjun Cave, Furong Cave, Dongge Cave, and Shenqi Cave generally showed a negative trend, which contrasted with the positive δ18O trend observed in the stalagmite from Feilong Cave (this study) (Fig. 3A,E,F,G,H).
In addition, by comparing the FL2102 record (this study) with δ18O records from stalagmites in the middle and lower reaches of the Yangtze River, including Heshang Cave (110° 25′ E, 30° 27′N)15, Sanbao Cave (110° 26′ E, 31° 40′N)58, and Lianhua Cave (109° 32′ E, 29° 29′N)59, certain trends emerged. During the MWP, the δ18O records from the FL2102 stalagmite exhibited similarities with Heshang and Sanbao records (Fig. 4A–C). All three stalagmite δ18O records showed generally negative values compared to the baseline value of zero, indicating an enhancement of the ASM, accompanied by interdecadal climate oscillations reflected in positive δ18O anomalies. In contrast, during the LIA, the δ18O values were predominantly positive relative to the baseline value of zero, signaling a weakening of the ASM11,31,39,40.
Comparison with stalagmite records from the middle and lower reaches of the Yangtze River. (A) Feilong Cave (FL; this study); (B) Heshang Cave (HS)15; (C) Sanbao Cave (SB)58; (D) Lianhua Cave (LH)59. Dating points and errors for each record are represented by colored dots and error bars, respectively. Pink and gray bands represent the Medieval Warm Period and the Little Ice Age, respectively. The black dashed line denotes the benchmark value of zero in the Z-scores analysis of each record.
During the MWP, the overall trend of δ18O values of stalagmite from Feilong Cave was generally consistent with those from Dandak Cave and Jhumar Cave in the Indian monsoon region. The δ18O values of these stalagmites were predominantly negative, indicating a consistent climatic trend between the East Asian and Indian monsoon regions during the MWP, with strengthened summer monsoon (Fig. 5)60,61. During the LIA, the overall trend of δ18O values of stalagmites from Feilong Cave, Dandak Cave, and Jhumar Cave was predominantly positive, indicating weakened summer monsoon (Fig. 5)60,61. The stalagmite record from Feilong Cave showed consistent variations with the ISM on decadal to centennial time scales, indicating that the stalagmite δ18O from Feilong Cave primarily recorded the climate signal of the ISM, consistent with the observations that for the summer precipitation in southwest China, mass of the moisture originate from Indian Ocean62. The differences among the three records during the LIA may be due to differences in dating points and resolutions of each record63.
Comparison with stalagmite records from the Indian monsoon region since the middle ages. (A) Feilong Cave (FL; this study); (B) Dandak Cave (DK)60; (C) Jhumar Cave (JHU)61. Dating points and errors for each record are represented by colored dots and error bars, respectively. Pink and gray bands represent the Medieval Warm Period and the Little Ice Age, respectively. The black dashed line denotes the benchmark value in the Z-scores analysis of each record.
During the MWP, the temperature in northern hemisphere increased11,17, the ASM strengthened, particularly enhanced the long-range southwest moisture transport (carried by the ISM)11, resulted in the northward shift of the rain belt in the monsoon region of China, and finally increased precipitation in north China11. According to the Rayleigh fractionation principle64, long-distance transported water vapor may undergo a series of precipitation processes upstream along the transport path, leading to the continuous separation of isotopic systems, resulting in lower δ18O values of precipitation in downstream areas65. During the MWP, the δ18O values of stalagmites from Huangye Cave, Wanxiang Cave, and Wuya Cave in northern China (Fig. 3B–D), as well as from Heshang Cave, Sanbao Cave, and Lianhua Cave in the middle and lower reaches of the Yangtze River (Fig. 4B–D), were somewhat negative. This indicating the strengthening of the ASM, northward shift of the rain belt in the monsoon China, and increased precipitation in north China. In contrast, the overall δ18O values of stalagmites in the south region of China, including Shijiangjun Cave, Furong Cave, Dongge Cave and Shenqi Cave, were relatively positive during the MWP (Fig. 3E–H), attributed to the northward shift of the summer monsoon rain belt and/or relatively reduced precipitation in the south, coupled with land warming enhancing the evaporation effect10,66, leading to drying in the south and strengthening of local water cycles10,66. Since local water vapor mainly originates from the evaporation of inland rivers and lakes enriched in stable isotopes, the precipitation δ18O values produced by this type of water vapor are biased65. Therefore, during the MWP, a “south dry-north wet” pattern was observed in the monsoon China.
During the LIA, the temperature in northern hemisphere decreased7,11, the ASM weakened, southwestern moisture transport diminished, the rain belt in the eastern monsoon region of China shifted southward, and precipitation increased in southern China11. During the LIA, the δ18O values of stalagmites in northern China showed a positive trend (Fig. 3B–D), significantly biased toward the δ18O values during the MWP. At the same time, the δ18O values of stalagmites in the middle and lower reaches of the Yangtze River also showed a significant positive trend compared to the MWP (Fig. 4), indicating a weakening of the ASM during the LIA. In contrast, although stalagmite δ18O values in southern China showed a positive phase during the LIA, the stalagmite δ18O values in south China were negative on the whole (Fig. 3E–H), attributed to the southward shift of the rain belt and relatively increased precipitation in the south7,66. Additionally, during the LIA, sea surface temperature in the Western Pacific Warm Pool were lower than normal, convection activity between the Indochina Peninsula and the Philippines weakened, and the Western Pacific Subtropical High system may have anomalously shifted southward63,66, resulting in prolonged retention of the rain belt in southern China and increased monsoonal rainfall in this region67. Therefore, during the LIA, a “south wet-north dry” pattern was observed in the monsoon China.
The differences in precipitation patterns between north and south regions have been confirmed by other climate records in monsoon China. For example, the pH values of Tsuifong Lake (121° 31′ E, 24° 30′ N) along the southeast coast of China were consistently lower than average during the MWP (800–1300 AD), but noticeably increased during the late stages of the LIA (1500–1900 AD), indicating a relatively dry climate during the MWP and increased precipitation during the late LIA (Fig. 6A)68. The total organic carbon (TOC) records from Huguangyan Lake (110° 17′ E, 21° 90′ N) in Guangdong province showed that TOC values were below average during 800–1300 AD, suggesting a dry climate during the MWP; however, from 1300 to 1720 AD, TOC values were consistently above average, indicating a wetter climate during the LIA (Fig. 6B)69. Pollen-based reconstructions of rainfall from Dajiu Lake (110° 00′ E, 31° 34′ N) in central China show a relatively dry climate during the MWP and a wetter climate during the LIA (Fig. 6C)70. Sediment grain size records from Lugu Lake (100° 47′ E, 27° 42′ N) in southwestern China indicated that grain sizes were smaller than average during 800–1300 AD but larger than average during 1300–1750 AD, suggesting a dry climate during the MWP and a wetter climate during the LIA (Fig. 6D)71. Finally, sediment grain size records from Erhai Lake (100° 10′ E, 25° 53′ N) in Yunnan province also indicated a relatively dry climate during the MWP and a wetter and unstable climate during the LIA (Fig. 6E)72.
Comparison of paleoclimate records in southern China since the Middle Ages. (A) Tsuifong Lake68; (B) Huguangyan Lake69; (C) Dajiu Lake70; (D) Lugu Lake71; (E) Erhai Lake72; (F) Feilong Cave (FL; this study). The red dots and error bars at the bottom represent the dating points and age errors of stalagmite FL2102. The pink and gray bands represent the Medieval Warm Period and the Little Ice Age, respectively. The black dashed line in each of A, B, C, D and E represents the average values of the various record indicators presented, whereas in F, the black dashed line denotes the benchmark value of zero in the Z-scores analysis.
The conclusions drawn are primarily based on changes observed in paleoclimatic records. In Feilong Cave, located in Guizhou, Southwest China, the δ18O values of stalagmites indicated a negative shift during the MWP and a positive shift during the LIA. This suggests that the region was relatively more humid during the MWP and drier during the LIA. This pattern is contrary to the trend observed in other paleoclimatic records from Southern China, which indicated a drier climate during the MWP and a wetter climate during the LIA (Figs. 3 and 6). The observed discrepancy may be attributed to geographic differences in the cave environments63. By comparing climate proxies in Southwest China, the stalagmite δ18O from Dongge Cave (25° 17′ N, 108° 05′ E) in Guizhou Province exhibited high-frequency oscillations on decadal to centennial scales centered around the baseline value of zero during the MWP, with two distinct positive phases observed during the LIA: 1350–1440 AD and 1500–1700 AD (Supplementary Fig. 2A)32. These positive phases coincided well with the two distinct positive phases recorded by stalagmite δ18O from Shenqi Cave (28° 56′ N, 103° 06′ E) in Sichuan Province: 1350–1440 AD and 1550–1600 AD (Supplementary Fig. 2B)30. Both cave stalagmite δ18O records showed positive phases corresponding to two notable weak Asian summer monsoon events in Chinese history: the “Weak Summer Monsoon Event at the End of the Yuan Dynasty” and the “Weak Summer Monsoon Event at the End of the Ming Dynasty”30. The grain size of sediments from Lugu Lake (27° 42′ N, 100° 47′ E) in Yunnan Province was smaller than the average during the MWP and larger than the average during the LIA from 1300 to 1750 AD, indicating a drier climate during the MWP and a wetter climate during the LIA (Supplementary Fig. 2C)71. The grain size of sediments from Erhai Lake (25° 53′ N, 100° 10′ E) in Yunnan Province was smaller than the average from 900 to 1450 AD, suggesting a drier climate in the region during the MWP and the early LIA; after 1450 AD, the grain size of Erhai Lake sediments gradually increased with larger fluctuations, indicating a wetter and more unstable climate in the region (Supplementary Fig. 2D)72. During the mid-to-late LIA from 1500 to 1900 AD, the grain size records from Lugu Lake and Erhai Lake showed out-of-phase variations, reflecting the complexity of precipitation changes in Southwest China (Supplementary Fig. 2C,D)63.
Additionally, reconstructions of precipitation patterns in Northern and Southern China since the MWP, based on various proxy indicators, might show some degree of uncertainty and variability. This uncertainty arises from factors such as the number of records, spatial distribution, sensitivity to climate responses, dating methods, dating errors, and resolution30,39,40,63. Southwest China was influenced by a dual monsoon system comprising the Indian summer monsoon and the East Asian summer monsoon63. The intricate precipitation patterns observed in this region were deeply sculpted by its diverse topographical and landform characteristics, with significant variations in terrain configuration, altitude gradients, and slope orientations playing pivotal roles in generating notable disparities in precipitation across Southwest China63. Therefore, acquiring high-precision, high-resolution records that cover a larger geographic area is critical and urgent for improving paleoclimatic reconstructions on decadal to centennial timescales in both Northern and Southern China30,57,62.
In summary, the climate patterns in the monsoon China have exhibited an anti-phase relationship between the north and south since the Medieval period. During the MWP, a “south dry-north wet” pattern was observed, while during the LIA, a “south wet-north dry” pattern prevailed. It is worth pointing out that during the LIA, the precipitation pattern in southern China, including the middle-low Yangtze River basin, southeast China, southwest China, and south China, is still presented spatial variability based on existing studies40,57,73. Furthermore, high-precise and multi-proxy records are essential to deeply reveal the changes in regional precipitation and hydrological conditions.
Forcing for the ASM
Multiple paleoclimate records from the Asian monsoon region indicated that during the MWP, the ASM showed an overall strengthening trend in response to the rising temperature and summer solar insolation in the Northern Hemisphere. While during the LIA, the ASM exhibited an overall weakening trend, corresponding to the declining temperature and summer solar insolation in the Northern Hemisphere (Fig. 7A,B). On a millennial scale, the ASM strengthened with increasing solar insolation and weakens with decreasing solar insolation27,28,66,74.
The driving mechanisms for the evolution of the Asian summer monsoon since the Medieval Warm Period. (A) Total Solar Irradiance (TSI)74; (B) Northern Hemisphere Temperature Anomaly (NHTA)4; (C) Titanium content in the Cariaco Basin81, indicating the north-south movement of the ITCZ; (D) Intensity of the Asian summer monsoon82; (E) ENSO Index80; (F) Atlantic Multidecadal Oscillation (AMO) Index95; (G) δ18O values of stalagmite FL2102 (this study). The red dots and error bars at the bottom represent the dating points and age errors of stalagmite FL2102. The pink and gray bands represent the Medieval Warm Period and the Little Ice Age, respectively.
Solar insolation is a crucial external factor driving changes in the ASM17,55, it produces wind by affecting atmospheric circulation, with the atmospheric circulation over the tropical Pacific Ocean further transmitting and amplifying the effects of solar insolation changes17,55. The tropical Pacific Ocean serves as a vast heat reservoir and a significant source of atmospheric moisture27,28. Its variations not only affect climate changes in tropical regions but also impact the climate of mid-high latitudes through atmospheric circulation systems like the Hadley circulation27,28. In the climate system of the tropical Pacific, the two hydrological systems that have the greatest regional and global impact are the ITCZ and the ENSO47,75.
Changes in solar insolation led to the north-south movement of the ITCZ47,55. During the MWP/LIA, with the increase/decrease in temperature in the Northern Hemisphere7, the land warmed rapidly/slowly as the summer Northern Hemisphere received more/less solar insolation, enhancing/weakening the contrast in thermal properties between land and sea and causing the ITCZ to shift northward/southward67,76,77,78. Precipitation in the Asian monsoon region is significantly influenced by the meridional transport of water vapor via the Hadley circulation11,79,80. When the ITCZ moves northward, the ascending branch of the Hadley circulation strengthens, the ASM strengthens or moves northward accordingly11,79,80. Conversely, when the ITCZ moves southward, the ascending branch of the Hadley circulation weakens, the ASM weakens or moves southward (Fig. 7C,D)11,79,80.
There is a significant positive correlation (r = 0.55, p < 0.01) between the δ18O of stalagmite FL2102 and the titanium content records from the Cariaco Basin in Central America, which indicated the north-south movement of the ITCZ81. During the MWP, the titanium content increased from 0.15% to around 0.3%, indicating a northward shift of the ITCZ, corresponding to the lighter δ18O of stalagmite FL2102 and the strengthening of the ASM (Fig. 7C,D,G)11,31,82. During the LIA, the titanium content decreased from around 0.3% to around 0.1%, indicating a southward shift of the ITCZ, corresponding to the heavier δ18O of stalagmite FL2102 and the weakening of the ASM (Fig. 7C,D,G). Therefore, on decadal to centennial timescales, the north-south movement of the ITCZ responds to solar insolation, thereby influencing the strength of the ASM.
Furthermore, ENSO activity is another important factor influencing the variability of the ASM48,80,83,84. During the MWP, ENSO activity was predominantly in a negative phase characterized by La Niña48,80,83,84, corresponding to lighter FL2102 δ18O values and a stronger ASM (Fig. 7D,E,G). In the LIA, ENSO activity was generally dominated by the positive phase of El Niño48,80,83,84, corresponding to heavier FL2102 δ18O values, indicating a weaker ASM (Fig. 7D,E,G). ENSO activity primarily influences the variability of the ASM by modulating the ocean-atmosphere circulation patterns. During El Niño events, the weakening of the Walker circulation and the reduction in the intensity of the ascending branch led to an eastward shift of the Walker circulation away from the Western Pacific Warm Pool19,48,80,85. Meanwhile, the strengthening of the Western Pacific Subtropical High extends westward to the southwestern region of China65. These changes in circulation patterns result in a weakening of the ASM, corresponding to heavier δ18O values in stalagmites (Fig. 7D,E,G); the opposite occurs during La Niña events (Fig. 7D,E,G)48,86,87.
In summary, the most important external factor regulating the variability of the ASM is solar insolation, which dominated the cyclic variations of the monsoon. The influence of tropical ocean-atmosphere circulation on the amplification and transmission of solar activity changes leads to the diversity of ASM variations, exhibiting frequent climate oscillations at decadal scales. Within the tropical Pacific climate system, the northward and southward movement of the ITCZ, as well as ENSO activity, are important factors influencing the variability of the ASM.
AMO, PDO, and ASM
The AMO refers to the quasi-periodic cold and warm anomalies in sea surface temperature in the North Atlantic, occurring over 60–70 years88. The primary cycle of the FL2102 stalagmite δ18O record, approximately 64 years (Fig. 8A), aligns with the typical AMO period of 60 to 70 years88, underscoring the close relationship between the Atlantic ocean-atmosphere oscillation and the ASM. AMO phase transitions have significant impacts on both global and regional climates. As a major multidecadal sea surface temperature mode, the AMO is a significant driver of changes in the ASM89.
Wavelet analysis for the δ18O and δ13C of stalagmite FL2102. (A) Wavelet analysis plot of stalagmite FL2102 δ18O; (B) Wavelet analysis plot of stalagmite FL2102 δ13C. Wavelet analysis was conducted using Matlab, where spectral power (variance) is represented by colors ranging from blue (weak) to red (strong), and the black curve indicates the 95% confidence level90. For Matlab calculation methods, refer to http://noc.ac.uk/using-science/crosswavelet-wavelet-coherence .
The AMO phase transition can lead to significant climate changes across various regions of the Northern Hemisphere and has a notable impact on the interdecadal variations of the ASM through several pathways91,92,93,94. The first is through the air-sea feedback mechanism in the tropical western Pacific–Indian Ocean. Coupled ocean-atmosphere simulation experiments indicate that a positive AMO phase induces warm anomalies in the tropical Western Pacific and oceanic continents, resulting in increased local precipitation91. Additionally, the convection activities and atmospheric heating in these regions can trigger anticyclonic circulation anomalies in the lower troposphere of the Northwest Pacific, leading to a westward extension of the Western Pacific Subtropical High and thereby strengthening the ASM91. In contrast, a weakened Atlantic meridional overturning circulation cools the sea surface temperatures in the North Atlantic, consequently weakening the ASM through air-sea feedback mechanisms92. The second is to change the atmospheric temperature of the Eurasian troposphere. A positive AMO phase raises tropospheric temperature over the Eurasian continent, increasing the thermal contrast between the Asian landmass and the surrounding oceans, which in turn strengthens the ASM93. The third aspect pertains to the propagation dynamics of high-latitude atmospheric wave trains within the context of modulation. Across interannual timescales, a positive phase of the AMO has the capacity to elicit Rossby wave patterns, which propagate downstream in an eastward direction originating from the North Atlantic. This results in anomalous low-pressure systems over northern Asia and high-pressure systems over southern Asia, enhancing southerly winds and northward moisture transport in the region, thereby reinforcing the ASM94.
By comparing the AMO index with the intensity of the ASM, it is observed that during the MWP, the ASM was strengthened, corresponding to a positive AMO phase. In contrast, during the LIA, the ASM weakened, aligning with a negative AMO phase. This further indicates that AMO phase transitions significantly impact ASM variations on interdecadal timescales (Fig. 7D,F)89,90,91,92,93,94,95.
The interaction between the AMO and the Pacific significantly influences on the variability of ASM96. The decadal modes of sea surface temperature in the North Atlantic and North Pacific are two of the decadal signals in the global oceans and are significant factors driving decadal changes in ASM96. When the AMO and the PDO are in opposite phases, their effects align and reinforce each other, resulting in significantly stronger or weaker ASM (Supplementary Fig. 3a,b)95,97,98. Conversely, when the AMO and PDO are in the same phase, their effects counteract each other, leading to slightly stronger or weaker ASM (Supplementary Fig. 3c,d)95,97,98.
Therefore, the decadal variability of ASM is influenced not only by the AMO, but also by the phase combinations of the AMO and PDO89,90,91,92,93,94,95,96,97.
Stalagmite δ13C and the evolution of regional ecological environment
There is a significant positive correlation between the δ18O and δ13C values of stalagmite FL2102 (r = 0.63; p < 0.01) (Fig. 2). The covariation of stalagmite δ18O and δ13C records at centennial scales has been confirmed in multiple stalagmite records within the Asian monsoon region32,39,47,51,77,87,99. Cross-wavelet analysis of δ18O and δ13C revealed similar dominant periodic variations at decadal to centennial scales (significant cycle of 64 years) (Fig. 8), potentially driven by the same controlling factors. Increasing, studies suggested that the positive correlation between stalagmite δ18O and δ13C records cannot be simply explained by kinetic fractionation during stalagmite deposition39,40,43,100,101. Even if kinetic fractionation exists, the climate signals contained in stalagmite δ13C records would not be completely obscured26,39,40,43,44,101, because the biological processes and inorganic processes which influencing on the changes of δ13C are directly or indirectly, closely related to the regional hydrological conditions42,50. The δ13C of stalagmite FL2102 may reflect regional ecological evolution under the influence of the ASM39,42,43,50.
Temperature records in China indicated warmer temperature during the MWP102 (Fig. 9A). During the LIA, the weakening effect of solar insolation due to volcanic ash entering the atmosphere led to colder periods5. Temperature during the LIA were about 0.5 °C lower than the average temperature during 1961–1990 3–4. The δ18O values of stalagmite FL2102 were lighter during the MWP and heavier during the LIA, reflecting an overall response to temperature changes (Fig. 9B). The δ18O records of stalagmite FL2102 oscillated frequently and presented a positive trend during the LIA, indicating a weakening and unstable ASM (Fig. 9B).
Comparison of stalagmite FL2102 δ13C with other climate records. (A) Temperature anomaly in China102; (B) δ18O record from Feilong Cave with Z-scores analysis (this study); (C) δ13C record from Feilong Cave (this study); (D) δ13C record from Shijiangjun Cave (SJJ)57; (E) δ13C record from Furong Cave (FR)49; (F) δ13C record from Dongge Cave (DG)32; (G) Historical population changes in China19,103. The dating points and errors of each record are represented by dots and error bars of corresponding colors, respectively. Pink and gray bands represent the Medieval Warm Period and Little Ice Age, respectively. The black dashed line in each of C, D, E and F represents the average values of the various record indicators presented, whereby in B, the black dashed line denotes the benchmark value of zero in the Z-scores analysis.
Both in MWP and LIA, the variations in δ13C values of stalagmite FL2102 responded to changes in δ18O values, even in some detailed structures (Fig. 9B,C). The covariance between the δ18O and δ13C records of stalagmite FL2102 indicated a close connection between stalagmite δ13C changes and climate variations. The positive trend in the δ13C values of regional stalagmites during the LIA suggested that the decrease in temperature and weakening of the ASM further exacerbated the degradation of the karst ecological environment in southwest China (Fig. 9C–F)32,49,57.
However, there are spatial differences between stalagmite δ13C records in southwest China and temperature records as well as ASM records, especially during the LIA (Fig. 9). The similarity between the stalagmite δ13C values of Shijiangjun Cave and Feilong Cave shows negative deviations during the MWP and positive deviations during the LIA (Fig. 9C,D)57. The δ13C values of Furong Cave stalagmite also exhibited characteristics of negative deviations during the MWP and positive deviations during the LIA; however, around 1150 AD, the sudden positive deviation in the stalagmite δ13C values of Furong Cave is significantly different from the gradual positive deviations in Feilong Cave and Shijiangjun Cave stalagmites (Fig. 9E)49. Meanwhile, the δ13C values of Dongge Cave stalagmite showed relatively consistent fluctuations during the MWP and LIA periods, without a significant positive trend around 1300 AD as preserved in other records. There was only one noticeable positive deviation during the period of 1500–1600 AD in Dongge record (Fig. 9F)32. This may be because the surface vegetation type in the area of Dongge Cave belongs to karst forests, maintaining a relatively humid surface state. Only under particularly severe disturbances will the ecological environment degrade significantly, resulting in noticeable positive deviations in stalagmite δ13C values32,53.
During the period from 1000 to 1200 AD, temperature records in China and the stalagmite δ18O in Feilong Cave maintained relatively stable fluctuations, indicating a relatively warm climate at that time (Fig. 9A)102, accompanied by a stronger ASM (Fig. 9B). However, the δ13C values of stalagmites in Feilong Cave, Shijiangjun Cave, and Furong Cave showed a gradually increasing trend (Fig. 9C,D,E)49,57. The positive trend in δ13C values of stalagmites correlated with the increasing trend in population (Fig. 9G)19,44,103. Additionally, during the period of 1700–1800 AD, temperature and ASM variations were relatively stable (Fig. 9A,B)102, while the δ13C values of stalagmite in Feilong Cave continued to be heavier (Fig. 9C), corresponding to rapid population growth (Fig. 9G)19,44,103. These comparisons indicated that during the MWP and LIA, besides climatic conditions104,105,106,107,108,109,110, human activities were also important factors influencing ecological changes in the region11,19,39,40,44,57. Population growth and increased land cultivation intensified ecological degradation11,39,40,44. During the MWP, specifically in 1127 AD, the famous historical event “Jingkang Event” occurred in China, leading to the fall of the Northern Song Dynasty40. After the “Jingkang Event”, the political and economic center of ancient Chinese civilization shifted from the north China to the south China for the first time. To be precise, the capital was moved to Lin’an Prefecture (present Hangzhou city), in the south of the Yangtze River. Thereafter, a large-scale migration of the Chinese population moved to the Yangtze River basin40, including the area where Feilong Cave is located. Compared to the period of 800–900 AD, the total population of China increased to about four times its original size from 1000 to 1300 AD (Fig. 9G)19,103. To alleviate population pressure and meet the needs of the rapidly growing population, continuous land cultivation was necessary, leading to worsening surface ecological conditions. Weakening of the summer monsoon, extensive deforestation due to population migration/increase, reduction in vegetation cover, and increased agricultural activities contributed to the decrease in soil CO2 production due to reduced respiratory and organic decomposition processes in plant roots, which was also one of the reasons for the positive δ13C values of stalagmite in Feilong Cave during the LIA11,19,51,111,112. Additionally, the introduction of C4 crops such as maize (with more positive δ13C values) may also have contributed to the positive trend in δ13C values of stalagmites11,19,39,40,44,113.